The
Physiology of Transport Substances in the Blood (Sodium)
By Professor
Marcel Uluitu, M.D. Ph.D.
Spiru Haret University
Bucharest, Romania
Co-Authored
by Diana Popa (Uluitu), M.D.
Department of Microbiology, Immunology
and Molecular Genetics
University of Kentucky, Lexington,
Kentucky, USA
[Editor’s Note: This paper is presented as Part IV of a
series of chapters from the new book “The Physiology of Transport Substances in
the Blood (Sodium)”; subsequent chapters will be featured in upcoming issues of
this Journal. This segment features Chapter Five]
Chapter 5
Endocellular
Compartment
5.1.
Short presentation.
It consists of all parenchymal cells and of those supporting the body. Cell
(Hooke, 1665) is "the unity of biological activity capable of
autoreproduction in an environment free of other systems alive" in the
current acceptance (Loewy and Siekevitz, 1963 cit. 68), an extension of the
cellular theory of Schleiden and Schwann, 1935 .
The cell is bordered by a polarized membrane with a
selective permeability which separates it from the extracellular environment.
Its content - cytoplasm - is divided by endocellular membranes in which are
included organic cellular and genetic centers, the nucleus.The cell organites
include mitochondria, Golgi apparatus, lysosomes, plastides, various pigments,
vacuoles, endoplasmic reticulum, ribosomes, cytoplasmic connections,
microtubules. The Eukaryota membrane (68) is a thin ,
non- rigid structure , to cover the cell and mediates its interaction with
cellular environement (71). It is composed of ectoplasm, fine-grained superficial
layer and more
consistent endoplasm. Cell membrane has an average thickness of 7.5 A. It
consists of proteins, fat and carbohydrates. Plasmalema is a semi permeable
selective structure. At its level there take place processes of transport some
of them which do not need metabolic energy , as
diffusion, osmosis, facilitated diffusion and endergonic transport processes
(active transport, endocytosis, exocytosis). The cells are polarized, have a
potential electric and all respond to external stimuli through the cell
membrane.
5.2. Cell
types.
The cells are divided into: non excitable, excitable
and autoexcitable, classification which takes into account: (1) their ability
to respond to stimuli with a wave of depolarisation which is propagated along
excitable cell structures and (2) the
absence of propagation of depolarisation in non excitable ones.
Non excitable group are: hepatocytes, connective cells , epithelial cells,
glandular cells, macrophages, cillias cells, glia cells . These modify the membrane
potential by stimulation , but do not transmit the stimulus,
as a potential of action. The ionic flux has low amplitude and produces
specific cellular response.
Excitable cells include nervous and muscular cells. In these cells permeability
changes, depolarisation of membranes, result in large variations of
electrochemical potential
propagating as potential of action through these structures.
Autoexcitable cells are represented by the excitoconductor system (51, 116, 78) and by some neurons.The cells of the sinoatrial nodule
develop normal rhythm impulses: internodule ways nodule transmit stimuli to the
atrio-ventricular nodule.
From here , through the Hiss bundle, the stimulus passes into the Purkinje network.The autoexcitabile
nerve cells are located in the brain at
the level of the respiratory center.
Cells with similar function and form held together by a support matrix form a
tissue. Support matrix is secreted by parenchymal cells. Tissue cells having
the same function form part of an organ. In the tissue there is the
intercellular fluid as an element of structure and not as a floating medium for
cells .The intercellular fluid changes and is removed with difficulty,
which makes tissue not to be a suspension cell. Tissues are classified in
epithelial, connective tissue, muscle, nervous, blood, expressing their
dominant position.
5.3.
Excitability
Depends on the presence of various monovalent and bivalent ions. Analyses
regarding the relationship between living organisms and the environment have emerged already in the 17th
century. Glisson (1587 - 1677) notes its general characteristic, J.
Brown (1725 - 1788) also includes interactions between the internal organs.
At present, "excitability is defined as the ability to react with energy
consumption to changes in the external environment and internal cells (68, 78)
in their efforts to preserve their genetic condition."
The
response expresses stimulus conversion of energy to biological
energy and is in itself an
exchange of information between cells and the modification of the environment
elements.
To provoke a response, the stimulus must fulfil certain conditions: threshold intensity
producing the cell responses, to act for
a measurable time interval, to have a certain rapidity , to have a time
necessary to restore the structure of repetitive stimuli.
Excitability depends on the resting potential, on the intensity of
cellular metabolic processes, on the physical-chemical
status and on the chemical composition of the extracellular environment
(covered extensively in the previous chapters) and speed recovery of the
polarization.
Plants respond by: growth, Turgor (mimosa), tropisms
(phototropism). The animal body responds as a whole, coordinated and integrated
by the activity of the nervous system, ensuring promptness of response and that
of the endocrine system, through which the response to stimuli is
prolonged. Multicellular animals respond to stimuli by muscle
movements, secretions, emission of light, electrical discharge, complex reflex responses. In higher animals, the
reflex arc involving the nervous system by the receiving areas, nervous
pathways, peripheral effector areas, cellular responses by white blood cells,
ciliated epithelial cells, etc.. The adaptative response follows within the
limit of variation around a genetical average rate ,compatible with life.
The response of integral or fragmentary receiver
structures can be recorded and quantified as physical magnitudes (electrical,
mechanical, metric, vegetative, pressional, secretive, exocrine or endocrine,
metabolic processes intensity), psychological (psychic functions, psychic
aptitude, behavior, psychological adjustment, social performance, etc.). The integrated
answer involves the body's internal communication between the three spaces
separated by the known biological membranes. The parenchymal cells prepare
their envirommrnt (basic substance or their own extracellular matrix) of
reactive elements ,compatible to the functional
specific faetures (111, 21). The response of the body is developed with the
participation of mono-and bivalent electrolytes present in the three spaces, in
concentrations the characteristic to each.
5.4 Cell
membrane.
The cell membrane (5) represents cell cover, ensuring the separation of the
cytosole from the extracellular environment, mediates cell relations with
elements of the same type of information, through informative connections of
electrical nature and by metabolite products. At her level there are selective
transport and excitability processes. The cell membrane structure is dynamic, fluid,
semipermeable. All membranes are made up of lipids, carbohydrates and poteins.
Lipids are the major component. It is a film of 5nm thickness, with 2 layers,
where the proteins are included. .
Carbohydrates are located on the external side of the
membrane. They are rich in anionic groups and interact with the
elements of the interstitium.The membrane proteins ensure cell excitability and
at the same time the transport of soluble substances (56). Protein molecules
are dissolved in the two-layered lipids (5). They interact with the space
matrix. They ensure the transport of substances involved in excitability and the
connection with the cell cytoskeleton of neighboring cells (78). There are
especially glycoproteins (78, 131). It describes two types of proteins (78):
the integral proteins, which cross the entire thickness of the membrane, and
peripheral proteins (2, 5) attached to the surface of the membrane. Integral
protein are structural elements, forming ionic channels: for water, Na, K, Ca, acetylcholine.
5.4.1. Ionic channels.
Na channels (2, 185) have
approx. 0.35 nm. They open on both sides of the cell membrane
(Fig.16). On the external face there is the opening orifice called "por"
or activation gate.Orifice on the face
endocellular is called inactivation gate(3). The Na channel is formed (78) of a
single unique peptide, rich in carbohydrate residues and sialic acid with many
anionic groups (2.5). From the molecular point of view, the Na channel has
three homologue, unidentical subunits,
,
,
. The
group is involved in
the activation of the gate with the other two (78,11,6).
Na channels have two transmembrane domains, with the COO and Na groups situated
intracellularly (78). They are structured as a pattern made up of the helix
and a
sheet , antiparallel
bound by two bridges of SH. On the extracellular face there are glycosylates
residues rich in anionice sites , which explains (140)
the local accumulation of Na+ cations around the pole activator. The
inside of the Na channel is rich in anionic terminal polar groups of
aminoacids. They also have in their composition glicosylates and sugars : N-acetyl hexozamine, N-acetylneuraminic acid (55).
Na channels have the ability to select the Na+ ions from a mixture
of different cations. Na channels are surrounded by areas with hydrophobia
Lipid structures , by means of which some physical
properties of the channel are structured : viscosity, thickness, surface
tension, intervening in the dynamics of pores. Therefore ,
the Na channel appears as a complex
proteo-lipid structure with a specific role.
5.4.1.1. Methods of studying the Na channel.
The study of Na channel is made by micromethods (18)of patch clamping type , methods of fluctuations analysis, methods of
radioisotopes, biochemical, electrorecording , the use of neurotoxins , that
brought a note of accuracy because of the binding structures specific channels
contributing decisively to decipher their function. (31 ,
180, 196, 214, 119, 135).
5.4.1.2. Distribution of Na channels.
Na channels are widely distributed in biology, including plant cells and
bacteria (73, 123, 4, 207). In mammals (rats and
rabbits) they are present in the muscles in the neuromotor plate and tendons
(11, 73, 30, 7). It is also found in the pancreas,
brain, testicles, bone and less in the kidney and colon (25). In nervous tissue
it is on the neuron membrane, the some membranes and at the level of postsynapsis membrane , on synaptosoms , acting as excitator receptors (78).
5.4.1.3. Classification of Na channels
.
Ion channels are polymorphous from the structural and functional point of view and require the
use of different criteria for classification. First of all ,
affinity and the ability to transport
various ions can be taken into account: Na+, K+, Ca2+,
acetylcholine , for which they have high specificity. Na channels can be
classified acc. to their interaction with neurotoxins and with H (145, 180, 196). Some Na channels are blocked by amilorid
(18, 113, 114). Others are activated by veratridine (18, 122) and under the
action of amilorid decreases the height of the
potential action. These channels have significance in pathology
, in genetical arterial hypertension, cystic fibrosis, in
hyperaldosteronism (178). K channels are smaller and have no negative charge.
But they have the same topography as the Na (3, 5)
Channels of acetylcholine from the neuronal soma and
from the postsynaptic
region also allow the transport of
sodium.
Figure 16. Schematical representation of the Na channel. (78)
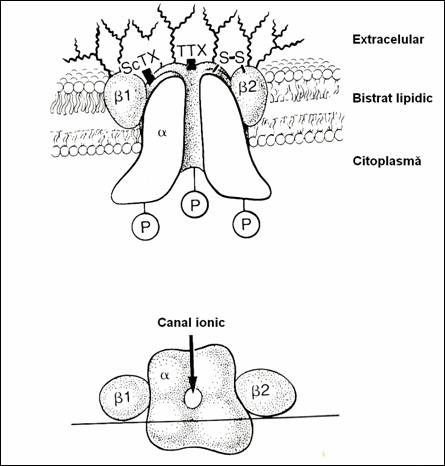
5.4.1.4. Na channel function.
Na channels initiate and lead the potential action (PA) in the axons, neurons,
muscle synapses. They work as receptors of environmental change through transitional
changes in Na+ conductance on the surface membrane (207).
They have an excitator role on postsynaptic membrane (78). The resting
potential of neuron soma is of-65 mV (lower than the peripheral fibers of - 90
mV) explained by the difference in the concentration of Na+: high in
the interstitial fluid (Figure 17) and very low ,
intracellularly . (78)
Due to the fact that the level of synapses , the Na channels allow
the faster movement of it and to neutralize a part of the neuron negative
charges and increases the potential of membrane from -65 mV to - 45 mV, which facilitates
the production of postsynaptic excitatory PA (78) . Channels-voltage Na and K
play a role in defining the rate of repolarization, adding itself to the action
of Na pump.
Figure 17.
Distribution of Na, K, Cl through the neurosoma membrane: PA origin on the soma
(78)
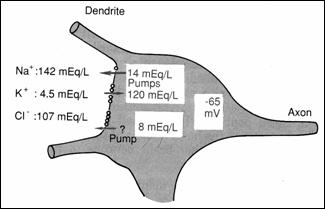
5.4.1.5. Na channels – pharmacological targets.
Ionic Na channels also function as pharmacological receptors with
therapeutic implications in local anaesthesia and anticonvulsant therapy (107).
They are molecular targets for neurotoxines: tetrodotoxin and saxitoxin (65,
180, 182) that block the channels of Na by acting unpon various segments (47). The
neurotoxins (Table 19) block the channels of Na, fixing themselves on anionic places instead of Na+
ions on the external face of the membrane near the activation orifice.
Table. 19: classes of toxins acting on the Na channel. (119)
Toxin pharmacological effects
Tetrodotoxin and saxitoxin (STX and TTX) blocks
currents of Na
Liposoluble molecule causes persistent
activation
of Na+ channels
veratridin
batrachotoxin
aconitin
grayanotoxin
Scorpion α toxin from North Africa, slows specific
North American and
polypeptide β toxin of Anemona. inactivation of
Na+.
Scorpion toxin from central and south American partially
blocks Na+
Centruroides suffusus (CSS II) Tityus serrulatus channels and creates partially (Tityus
Y). a new type of
channel
on the lower
potential
pyrethroids changes
the closing of
rapid Na channels .
ciguatoxin increases Na
permeability.
In Na channels of electrophorus electricus
, on polipeptides of inner canalului.The specificity of interaction of
neurotoxins with Na channels allowed their use as molecular targets (31). The action
of neurotoxins decreases in acid environment due to the antagonism
H+ (32), which remain fixed on polypeptide carboxyil groups, fixing
place of neurotoxins as well. STX is less influenced by the acid medium. The
most active compound is pyrethroid (123). Neurotoxins’ action is not influenced
by procaine.
5.4.2. Transport through membranes.
The cell membrane has selective properties for
different substances: nutritive, metabolic, functional specific substances
synthesized in the body. The conditions
regarding the transport of the substance show that it take place through pores existing in the membranes , through the spaces between the protein
molecules, between these and the ion channels and through ion channels , that
allow the passage of water and polarized hydrophilic substances (56). Non-polar
hydrophobic substances
are transported through areas rich in lipids of the membrane (25, 78)
Membrane permeability changes
under the influence of factors which modify excitability as well: stimulation, spontaneous
decrease of the resting potential, through the accumulation of Na+
in the extracellular space, by the action of pharmacological agents.
Transmembrane transport is made by active and passive processes (Fig. 16).
The passive transport of the
lipophylic substance is
done by separate portions of the membranes and the polar substances are transported
through the areas described.
Active polar substances transport is made by osmosis and diffusion, under the influence
of (136) of concentration and electrochemical gardients(106).
Diffusion and electrodiffusion takes place through the intermolecular
spaces and ion channels which they can interact with. The mechanism has
decisive importance in the passage of Na+. The facilitated diffusion is made by means
of transporters with which the transported substance interact (134). Such a mechanism
is known as "ligand gating" and the resulting molecule acts on an activated
gate of the Na channel, modifying the conformation and activating the gate. The
diffusion rate is equal to the resultant of the molecules passage in both
directions through the membrane. Transport of Na is facilitated (2) by the
richness of anionic charges presented above. Thus, it has been calculated,
that, in a single cycle, up to 7000 Na+ ion pass through a channel . The active transport
has a greater degree of specificity. It is consuming energy. In this transport
there participate pumps of Na.-K, the Ca mechanisms as well and countertransport.
In this type of transport an important part is played by the metabolism in
maintaing the membrane structure and activation of transport .
Figure 18. Conditions to determine the neural membrane
potential: A = when the membrane potential is caused only by diffusion K+;
B = when the membrane potential is caused by diffusion K+ and Na+,
C = membrane potential is caused by diffusion K+, Na+ to
which is added the Na+ , K+ (78) pump.
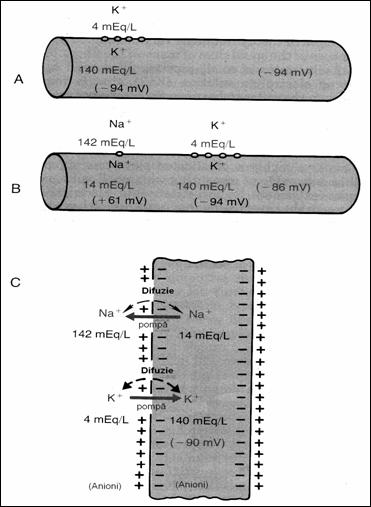
5.4.3. Membrane
potential.
Cell membranes are polarized. This is due to selective permeabilities and the
different environment on the two sides: the endocellular face is in contact with cytoplasm and
intracytoplasmic formations, the external face is in contact with the interstitial
matrix. The size of the potential is - 100 mV (Figure 15) depending on the cell
- animal or vegetal (68): the giant axon of mollusc or plant cells (Wallonia Holicystis).
The endocellular surface has negative charges and the extracellular surface , positive charges.
Table 20. Chemical composition of the
extracellular and intracellular (78) environement.
Compound extracellular intracellular
(mEq%o)
Na
140 10
K

40 140
Ca
24 0,0001.
Mg
1,2
58
CL
103 4
HCO
28 10
Phosphates
4 75
SO
1 2
Glucose
90 0-20
mg/dl
Aminoacids 30mg/dl
200 mg/dl
Cholesterol 0.5 2 - 95,0
Fosfolipids
- -
Neutral fats
- -
p0
35 20
PCO
46 50
pH
7.4 7,0
proteins
2/dl 16/dl
The
membrane potential is due to the concentration gradients of (Table 20) of each ion , chemical potential of the main electrolytes: Na+
and K+ (Bernstein, 1902). The resting potential (RP) is persistent
and is maintained as long as the cell is alive and active .It reaches "0" when ions are in balance .The
potential value of the membrane (PM) which prevents an ion diffusion through
the membrane is the Nernst potential.
5.4.3.1. Nernst
potential.
Its size is given by the ion concentration on both sides of the membrane and
expresses the trend direction of diffusion. Its value is calculated by eq. Of
Nernst at body temperature:
Eq.16. EMF (mV) =
61 log C
/ C
where:
C
and C
= concentration on faces internal and external membrane.
+ And - refers to cation, anion respectively
Iis value is = - 61 mV inside
The membrane potential (PR) of excitable cells in basic conditions has a value of - 90 mV in the
interior as compared to the interstitial fluid. By the activity of the Na-K pump there are actively transported in opposite
directions and their results an unequal distribution of the 2 (Table 20) (56)
on the two sides (Figure 16). The gradients of concentration are retained by
the activity of these structures, ion channels for Na and K of membrane proteins.
The K channel in the excitable membrane, in resting position is 100 times more permeable than the for
K+ than the Na channel for Na+.
The resting potential is maintained only by K ions (in excitable tissues).
The gradient of Na+ in the cell membrane
has a small role in maintaining or is invalid in maintaining the resting
potential (68). There results an added PR of - 86mV due to the great
permeability for K+ and non permeability for Na+. To this
is also added the contribution of the
electogenic Na, K pump (Fenn, Hodgkin, Keines) (Figure 16), out of which 4 Na+
ions are remove from the cell and 3 K+
ions pumped inside.
This resting potential becomes -90 mV. There is also added the low activity of Na+ ions (233) because of the interaction with extracellular
proteins. PM of - 90 mV is maintained by the fact that the gates activation of
Na channels are closed , so they are inactive,
although the inactivation gate is open, but ineffective.
|
There are other cationic gradients instead of K+, such as Ca2+.
Anionic contribution to the PR is given by their presence in the cell:
organic anions resulting from the metabolism (acetate, piruvat, milk, amino
acids, inorganic anion such as phosphate, sulphate) collectively called
"X-" and they not are outside the cell , rich
in Cl- (Table No. 20). Maintaining resting potential depends on
oxygen, thus on the energy consumption. In the absence of oxygen, K+
is lost but not completely , because of anaerobic glycolyte
processes, which may be stopped by specific toxins (iodacetic acid, fluorizin,
physical factors as well as the equilibrium
of Donan). They also maintain the Na+ ion pump ,
in their absence the K+ gradient disappering as well. The polarized
state and so the cell excitability is maintained by consumption of energy.The
complex functional membrane so structured and maintained is presented as a
metastable state which responds to the variations of environmental cells , at
a certain excitability threshold with a defined rate between the intensity
and duration of stimulus. The response is differentiated by the three types
of cells and metastable state is essential to produce action potential.
5.4.4.The Action Potential of excitable tissues
(Potential of Na).
The response of excitable and autoexcitabile cells to stimuli spreads through
rapid changes of the Resting Potential in the Action Potential. Action
Potential (AP) represents a temporary change of Resting Potential (RP),
following the initiation of a state of excitation, a change of selectivity
for ion membrane, with increased permeability to Na+ and increased
PM (Du Bois Reymond). Variation of potential is biphasic ,
like a sequence of differences of potential, with an exclusive role of Na
(78, 94, 3, 147). AP begins with a sudden change of RP, initiated under the
law "all or nothing", which is transmitted non-decremential (68,
78) as a wave of depolarisation (3) along the excitable structure (Figure
19). AP initiation takes place when RP increases by 1/5-1/3 of its max. value i.e from -90 mV to - 70 mV. The process requires
about 6 x 10 electrons. The value of -60 mV represents the threshold of
stimulation. At this time there take place the conformation changes of the
protein of the Na channel gates , activating and increasing
permeability for Na (89). The cation is attracted by the multitude of
negative charges of the Na channel gates which invades the cell. It results an increase
in membrane potential (MP) to induce the opening of more Na channels . Sodium
spreads through the aqueous environment of the channel and through the
interaction with local negative groups . The
direction of Na+ flow depends on MP and on the gradient of
concentration. After depolarization , ionic currents show three effects: (1) rapidly
increasing Na+ conductance, followed by (2), a slow decrease of Na+
conductance, so a process of inactivation of Na+ and (3) a slow
increase of the K+ conductance (Figure 19). These changes increase
the depolarisation reaching -100 mV and over. Depolarisation of the membrane
grows up to the positivation of the interior of the cell through
Na+ , which invades
it. During this period permeability for Na+ increases 500 up to
5,000 times, while permeability for K+ increases of only 30 times,
and K+ is lost in measurable quantities (19).
In addition to the changes mentioned at the point of
stimulation there take place other changes in membrane properties: the ohmic
resistance decreases from 1000 to 25 ohm / cm , it highlights the ionic exchange between the two surfaces
of the membrane, RP is cancelled, the membrane polarity changes . In this
phase there is a
steep increase in AP branch upwards (Figure 19).
AP is produced (68) exclusively by Na+ (48, 147) and it can be also
called potential of Na (Fig.20). MP is a potential of K+, Na
having a contribution of 1%. High speed of growth of AP involves a very large
number of Na+ ions in a short period of time. AP height
depends only on sodium in the extracellular medium(3,
68). The decrease of Na+ content is accompanied by a decrease in
AP height. Cations which penetrate the cell membrane diminish (45) the height of the spike.Some neurotoxins (Table
20) with high affinity for the gates activation channel of Na (32, 45, 108)
of nerves and miocite (118), but do not block the K gates . So, Na+
is the current conductor during the growth during AP. Replacing it with another
cation, which does not penetrate as fast as the membrane XNa (choline
clorhydrat) lowers the height of spike. The same effect is achieved in
proportion to the Na+ decrease . Gates function
is regulated by two mechanisms (113): (1) voltage gating. In this case
molecular conformation of gates respond to the
transmembrane electric potential. When there is an intense negative charge on
the internal face of membrane , the Na gates remain closely
closed. But when this charge is lost , the gate
opens. This mechanism is the main cause of AP. Some gate channel protein open
by other molecules bound with proteins. This induces a
conformational change in a protein molecule that opens or closes the gate.
This is called " ligand gating ” and the
substance becomes bound ligand. This is important in the synaptic trasmission
neuron-neuron and neuron-muscle for the acetylcholine transport
.
Figure
19. Changes of Na+
and K+ conductance during PA evolution.
Na+ conductance increases 5000 times at
the beginning of the PA and of K+
only 30 times in subsequent stages of the PA (78)
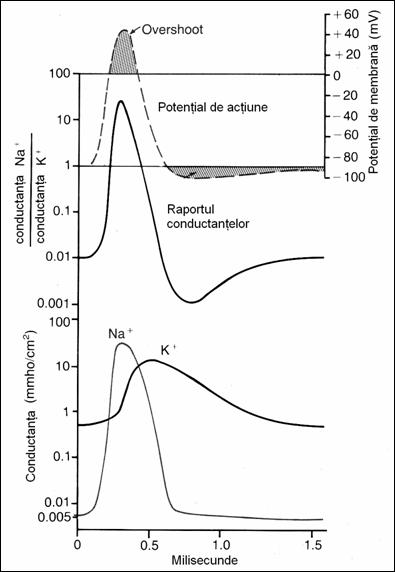
5.4.4.1. Restoration of resting potential.
The growth of AP with overshoot is followed by a series of events which have
membrane manifestations resulting in the restoration of RP. Restoration of ionic
polarization preceeds the fiber restoration. Membrane repolarization in RP is
slower than depolarisation and starts from the spike peak by activating Na
and K pumps, which transport active K+ to the cell, and the
membrane regains polarization. During resting, the voltage-gate of channel K
is closed and ion can not cross out. Increased MP from -90 mV to potential
"0", changing the voltage induces a slow change of conformation and
opens the K channel gate, located on the membrane endocellular face and thus K+
leaves the cell. The process is slower than the opening of the Na channels
activation gates. The same increase in voltage also closes the gate of the
inactivation of the Na channel and so it remains blocked, increasing the inner
positivation, even if K+ leaves the cell. So an increase of MP takes
place , closing the Na+ gate channels (3, 78 ) and lowers the penetration
of Na+ , but K+ continues to grow outside , because the
closing of K channels gates is slower . It can be concluded that
depolarisation and repolarisation membranes are under the control gate
voltage of Na channel (78), but the speed of repolarisation is provided by
the voltage gate of the channel K.
The right branch of the slower spike, (Figure 19, is produced by the inactivation
of Na channel by closing the activation gate and arresting Na+ in the cell. The channel
will reopen in the end , close to the fulfilment of repolarisation. At the same time, the K
pump function comes into operation ,coupled to that
of Na and restore RP (250), giving this process an endergonic character.
Figure 20. Flows of Na + and K + in the giant axon of sepia. (Shaded
columns: RP. White Columns: after stimulation by 100 cycle / sec) (3)
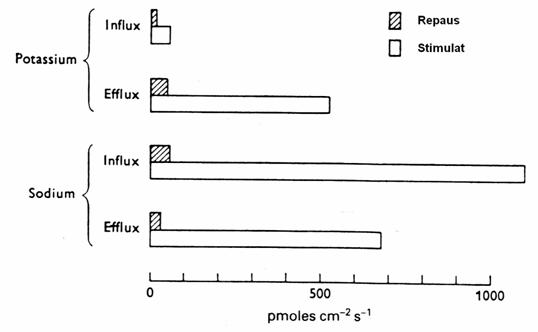
|
5.4.4.2. Dendrites potential.
Dendrite generally present a local unpropagated local decremented
potential. In these structures the Na+ entry and K+ exit are simultaneous
processes , differentiating themselves from the mechanism of ionic movements described in the
case of axons and muscles. The degree of response is determined by the number
of opened Na
and K channels and by the duration and size of each opened channel. So, these unpropagated potentials intervene
in the sensitive neurons function and in
synapses , allowing the passage of information and its integration.
5.4.4.3.The propagation of action potential.
The propagation of
the depolarization wave is made by current sources represented as movement of
Na+ (Hodkin and Huxley)along the
fiber. Propagation of AP is still dependent on Na+.
The theory of superficial nervous impulse conduction, the disturbed
local potential that induces electric potential on the surface. It is
more important in dendrites, where excitability is local, graded and decremential and AP appears by summation of stimuli.
|
The Saltatory theory of PA conduction is found again in
myelinisation fibers and the jump is made from a Ranvier node to another,
where the nerve membrane is in contact with the extracellular environment.
This is the preferred modality of transmission in central nervous system.
Sensory receptor cells respond to specific stimuli (45). Receptor stimulation
affects the frequency discharges, but not the height of impulse. There takes
place the frequency modulation of a single type of message. RP recovery is made
by energy consumption,from the phosphate macroergic
links. Polarization is regained during the relative refractory period, when
the membrane becomes again impermeable to Na+.
|
5.4.5.The action potential of autoexcitable
tissue.The role of Na.
The action potential of autoexcitable cells is studied
best on the excitoconductor heart system(sino-atrial
nodes, atrioventricular nodes, Hiss bundle, Purkinje network), smooth muscles,
some brain nuclei (neurons respiratory center).
Autoexcitability is
due to the incapacity of embryonic fibers and of autoexcitable neurons to maintain constant the RP membrane.
This is in relation to the time overlap and endogenous stimuli. During the diastole,
when the resting potential is maximum, depolarization is slow and continuous
until the discharge threshold of -60 m V -70 mV through changes of
conformation of proteins and probably through accumulation of Na+ in
the cell and the opening of Na gates takes place and the AP is started. The
potential is slow, has no overshoot, has a value of - 55 mV to a minimum - 60
mV, with a duration of 200 msec. In the heart there
are no fast Na channels, AP is not sensitive to tetrodotoxin but only to Mn2+ . In
this case , the role of Na+ is much
diminished , K+ having a greater role in the genesis and
transmission of AP. The mechnism of rhytmicity of sinoatrial node recognizes
the existence of an MP in diastole of -55 mV to-60 mV, negativity which is caused by the flow of
Na+ in the fiber. Depolarization initiated here is transmitted
directly to atriale muscle fibers. Polarization of the ventricular fibers is -
85 - 90 mV. In the myocard there are three types of membrane ion channels with a
role in the development of AP. (78): (1) fast sodium channels, (2) slow-calciun
sodium channels, (3) K-channels. The opening of fast sodium channels in a few
milliseconds is responsible for the rapid emergence of a spike AP observed in the
ventricular muscle caused by the rapid influx of Na in the fiber. AP plateau is
caused by ventricular slower opening of the channals of Na-Ca
, which delay tenth of a second . Finally , the activation of K channels increases and the
diffusion of a large amount of K+ outside of fiber and the return to RP takes place . The smaller negativity of
only RP-55mV explains why the fast Na
channels are more inactive, i.e, they are blocked because all the time MP remains less negative than -60 mV for
several msec , the gate of internal and inactive membrane and channels remain
closed. This , only the slow channels Na-Ca can be
opened and thus they can be activated and produce an AP. AP is thus slow and
produces the discharge of the ventricular
muscle. Autoexcitation of atrial sinus fibers also recognizes sodium as the main
element. Ions of Na+ tend to penetrate into the fibers of the node
membrane through multiple channels and the influx of positive charges increases
gradually during diastole. When it comes to the threshold voltage of -40 mV the
channels Ca-Na are activated and both ions quickly enter the cell causing AP.
So , the Na flow through through the fiber sinus is the cause of their
autoexcitation.This Na+ flow does not produce a continuous depolarization
, it occurs because of the inactivation of Na-Ca channels in 100 - 150 msec.
After opening or concomitant increase ,the number of K channel closed
increases. So , there ceases the function of Na-Ca
channels and there increases the outside diffusion of the cell K and AP ends . But the closure of K channels is delayed
, this is lost from the cell and thus increases
the negativity on the internal face of this membrane , which is hyperpolarized.
There follows the installation of RP - 55 mV-60 mV. The hyperpolarization is not
maintained continuously , because in the last few hundreds of a second after AP, more and more K channels close and the Na+ flow compensates cellular negativity and leads to -40 mV threshold of
excitation.
[Chapter 6 will be featured in the
upcoming March-April 2010 issue of this Journal.]
Professor Marcel Uluitu, M.D. Ph.D. began his
scientific activity in Physiology in 1953 at the Physiology
School (Medical
School) Cluj-Napoca,
in Romania.
He continued his scientific activity in Physiology in Bucharest at the
Institute of Physiology and Pathophysiology Daniel Danielopolu until 2004, at
which time he retired as Director of the Institute; he had held this position
since 1990. His research work includes studies of the central nervous system
physiology relating to the transmission of information in the nervous centers;
cerebral excitability, using methods, in their evolution, from the
determination on reactive isolated organ, to the most complex physical and
chemical methods, in the present. In his research of chemical mediation he
studied acetylcholine, serotonin, and their connection with the cerebral
metabolism.
Professor Uluitu has also investigated
cerebral tissue excitability, studying the structure modification of the
protein macromolecules, and the physiological and pathopysiological processes
in which are involved Sodium and Lithium. He implemented an original method for
physical and chemical processes which involve the chemic active sodium, in
normal processes and in the cerebral excitability dysfunctions, in human and in
experimental model (animal). These results of this work gave him the chance to
outline the chapter herein relating to the physiology of substances transport
in the blood. This is based on the physical and chemical interaction between
blood components.
His papers are included in the
collections of the U.S. National Library of Medicine and the U.S. National
Institute of Health. He is a member of the Romanian Academy
of Medical Sciences.
Dr. Diana Popa (Uluitu) is a
researcher in the Department of Microbiology, Immunology and Molecular Genetics
at the University of Kentucky in Lexington,
Kentucky, USA.
She attended Medical School at Vasile
Goldis University
in Arad, Romania,
and graduated in 1998; during summer breaks at medical school she volunteered
at the Institute
of Physiology D. Danielopopu
in the physiology and microbiology laboratories as well as in the clinic. After
completing medical school and a one year internship, she worked as a Research
Assistant at the Danielopopu Institute for three years before coming to the University of Kentucky. In addition to her medical
thesis, which focused on the actions of Lithium on the central nervous system,
since coming to the United
States she has published numerous additional
papers relating to Lithium and other substances. In continuing her on-going
studies, Dr. Popa is currently pursuing a Master’s degree in Public Health at
the University of
Kentucky.